A single E. coli placed in a sugary broth will divide into two genetically identical cells in twenty minutes. Another twenty minutes later, those two will grow and divide into four. Give those cells enough broth and room to grow and after about twenty-three hours there will be enough E. coli cells to fill an Olympic-size swimming pool. Wait another twenty hours and the mass of bacteria would equal the volume of the earth. Round it out to forty-eight hours and you’d have a ball of E. coli twenty-two times the size of Jupiter.
The scales possible with exponential growth are as incomprehensible as they are impossible. E. coli’s potential for exponential doubling is realized only in the highly controlled environment of a researcher’s test tube, where food is abundant and no other species are in the way. Even then, the time that bacteria can be expected to grow exponentially is inevitably limited—growth slows once nutrients start running low or the cells are too crowded to keep dividing further.
Biological growth scales to fit its context. There are no gas giants full of identical E. coli. More than one hundred years ago, D’Arcy Wentworth Thompson published On Growth and Form, a treatise on the mathematics of biological growth. In it, he summarizes this central maxim for biological scaling: “The effect of scale depends not on a thing in itself but in relation to its whole environment or milieu; it is in conformity with the thing’s ‘place in Nature,’ its field of action and reaction in the Universe.”
Biology’s ability to grow in relation to its environment—to grow ecologically rather than exponentially—is at the heart of what inspires biologists, engineers, and designers to work with organisms to build a new kind of technology. What if our technologies could grow and heal like living things?
What if concrete could be set by the metabolism of microbes, any cracks repaired in situ? What if factories were replaced with farms, growing new things that could be recycled back into the soil? What if, as MIT Media Lab director Joi Ito has proposed and further developed in a recent manifesto, “the role of science and technology [in the next hundred years] will be about becoming part of nature rather than trying to control it”?
People don’t multiply like E. coli, but put a few people together and you’ll quickly end up with more. Over the scale of millennia we’ve ended up with 7,589,052,176—the mind-boggling number of people on earth today, whose ability to satisfy basic needs for shelter, food, and warmth are always dependent on the availability and distribution of resources.
How we’ve grown to this number is a story about the industrial revolution, where the convergence of steam power, chemical development, mechanized production and the ideas revolution of the Age of Enlightenment led to great leaps and bounds in our capacity to create life-giving stuff—and therefore the conditions for populations to multiply. We are currently riding that exponential growth curve, and the UN expects this to peak at 11 billion by the end of the century. Within our current economic framework, that’s 11 billion consumers of life-giving resources, taking from living ecosystems without regard to the environmental milieu. Industrialization has put manufacturing on exponential curves that can be as incomprehensible as the growth of E. coli—and as devastating on a global scale.
The Re-industrial Revolution
Each of the 7,589,052,176 people currently on the planet uses an average of 1700 litres of water every day. This is partly for drinking and washing, but it’s mostly accounted for in the “embedded” water that’s needed to produce everyday products—the food we eat and what it took to grow or rear it, the clothes we wear, or the energy we use.
Industry is heavily reliant on water, and demand is expected to increase 400 percent by 2050. The textile industry in particular is a huge consumer and polluter of water: it takes 1083 gallons of water to produce one cotton t-shirt, twenty-six of which are needed for dyeing alone. Overall, textile dyeing uses enough water to fill 2 million Olympic swimming pools every year—a number that will continue to grow as more people buy more clothes and wear them for less time.
We live on one planet with finite resources and yet our mode of industrial production has managed to mimic the nightmare scenario of a devastating E.coli megaplanet. How might we transform the scale of industry to conform to its place in nature? How can we make technology grow ecologically rather than exponentially?
As a biologist (CA) and designer (NAC), we’ve approached the challenge posed by the need to scale technology within the bounds of nature by designing biology itself as the technology. Natsai’s recent work, for example, tries to minimize the amount of water used in textile dyeing, as well as the amount of pollution it produces. By growing a bacteria called Streptomyces coelicolor—a soil microbe that naturally produces pigmented molecules—directly on a textile, a colorfast pink, purple, or blue finish can be achieved without the use of toxic chemical additives. To dye a t-shirt using this method requires just 200 milliliters of water, 500 times less than current industrial techniques.
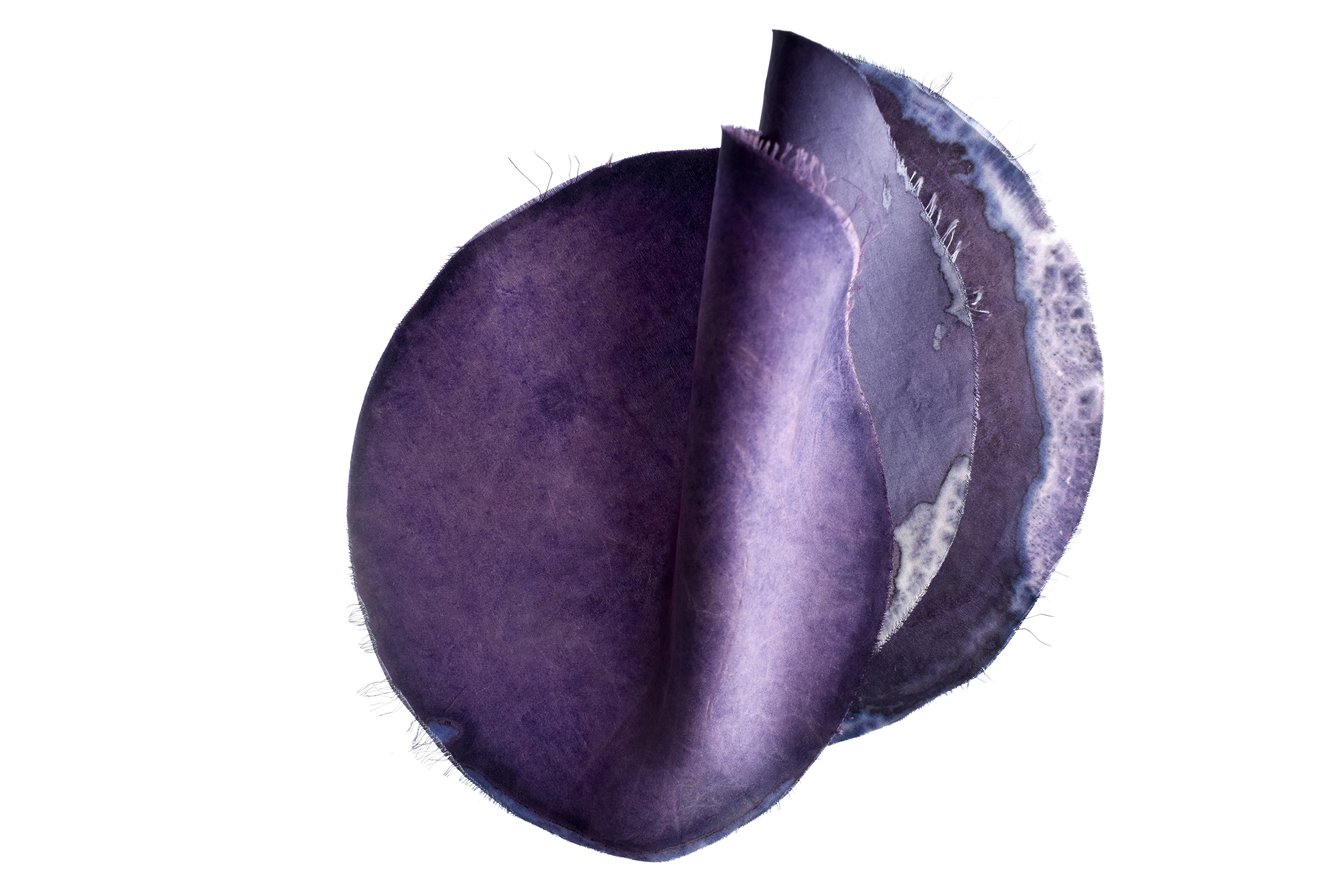
In this way, the bacteria becomes the coauthor in the creation of a new textile design. The designer no longer specifies all the details of the final product, but rather brings together the conditions in which the living process can thrive and create.
Adjust the pH, and the color changes from pink to blue. Alter the amount of oxygen or moisture, and the intensity changes. Alter the genetic pathways of the cell, and new colors and conditional patterns can emerge. This notion of co-authorship marks a fundamental paradigm shift for the designer: a new kind of learning to live within one’s means. Rather than the traditional model of specify, procure, and transform, the designer learns to develop outcomes with a living system and within finite biological limits.
Processes like these point towards the possibility for a new era of biologically derived materiality. Rather than an old industrial model based on extracting resources from nature, they allow us to think about how to re-industrialize according to biology’s ability to grow and scale—to extract nature’s logic, harnessing biological processes for human production.
Such a re-industrialization might enable new aesthetic and technical opportunities, from microbially defined patterns to liquid leather. We might imagine microbially enhanced homes, e-readers made from specially engineered leaves, or laptops grown from sawdust and programmable fungus.
While these offer inspiring visions for a biological future, achieving this future requires more than just biology. New technology is not enough; the surrounding system has to change as well. Dyeing textiles using bacteria may use much less water and do away with toxic effluent, but the economic imperatives that structure the fashion industry threaten to limit this innovation’s impact. The reality that underpins the fashion industry is a “race to the bottom line” business model, calibrated to sell more units no matter the cost—human or environmental.
Moreover, when industry does make use of biological methods, the impact is often destructive. Biological manufacturing forced into a culture of industrialization has led to dire ecological consequences (clearcut forests, feedlots) and will only continue to do so if we adopt biological processes without adopting biological constraints. Scaling biology to meet the needs of 7,589,052,176 people therefore requires us to reconsider and redesign more than just what can be encoded in DNA.
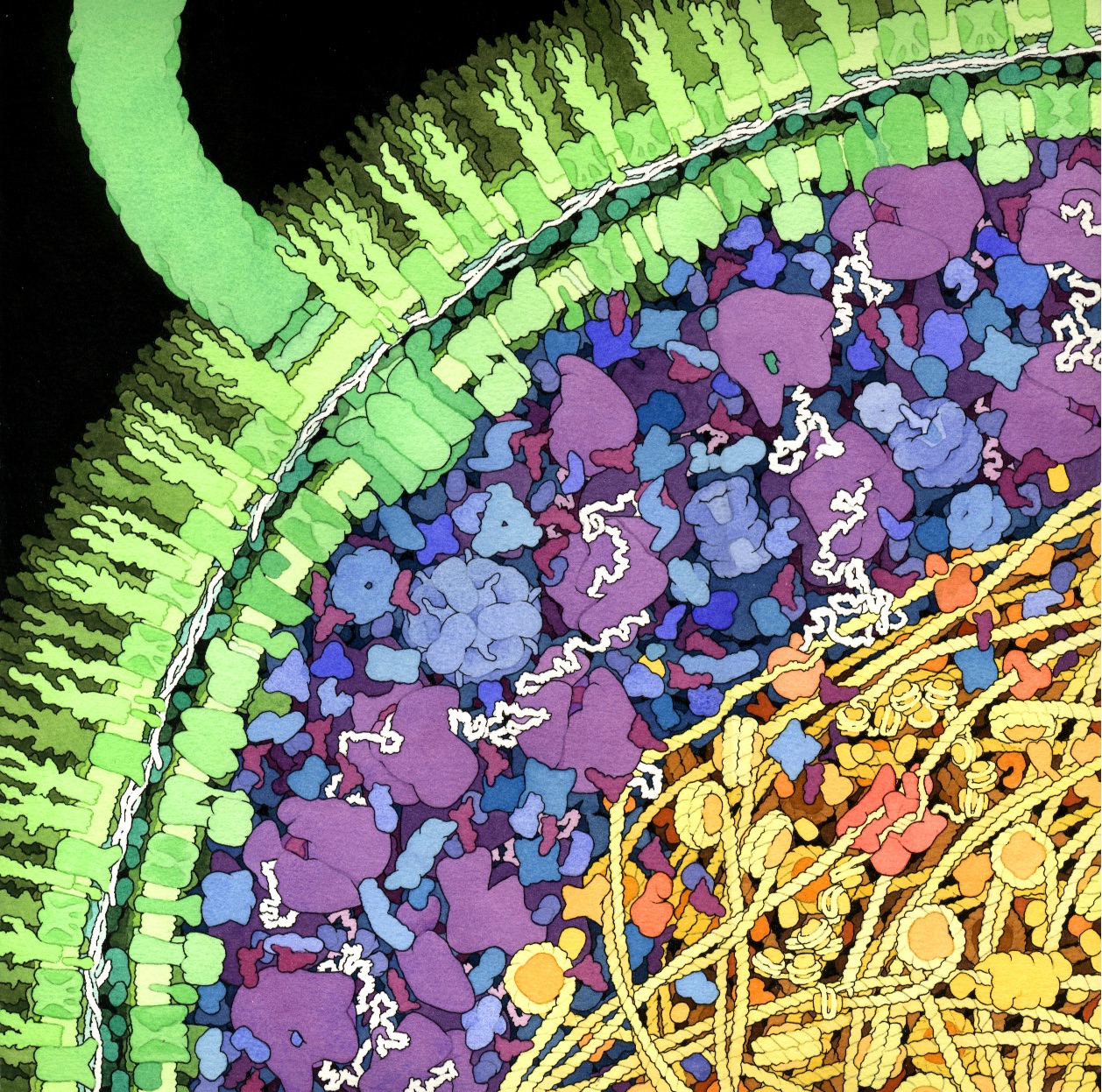
From Petri Dish to Planet
A single E. coli has a volume of about 0.7 cubic micrometers. More than seven trillion densely packed cells can fit into a teaspoon. Accessing the incomprehensible smallness of the molecular scale requires training, techniques, and tricks to bring the microscopic into focus.
At this laboratory scale, biologists and engineers have designed organisms that can produce medicines, fuels, materials, and chemicals—living, biological sources for all kinds of stuff that today is more likely to come from petrochemical sources. But scaling these processes from the lab into products that people can use has proven challenging. Despite microorganisms’ penchant for exponential growth in the right conditions, adapting these techniques to systems of industrial manufacturing takes much more than placing a cell in a vat and waiting for it to grow.
There are the technical challenges posed by the microbes themselves, unwilling to produce what we ask of them. But there are also challenges posed by scaling within the limits of overlapping contexts of ecosystem, economy, society, and planet. Biofuels, for example, are produced from renewable biological sources via bio-industrial processes, but the reality of how land use, government subsidies, fermentation, and the price of oil are entangled in its production mean that the jury is still out on whether biofuels are any better than gasoline when it comes to contributing to climate change.
Technology, as Ursula M. Franklin notes in The Real World of Technology, “entails far more than its individual material components. Technology involves organization, procedures, symbols, new words, equations, and most of all, a mindset.” A deep understanding of DNA, enzymes, and microbes—the individual material components—is necessary to scaling biological technologies. But so too is an understanding of chemical engineering and process development, environmental science and life cycle analysis, sourcing and supply chain management, economics and market dynamics, politics and regulatory governance, sociocultural forces, consumer needs and perception, product design, and marketing.
No one person can see across all these scales, from the molecular to the global. We need organizations and language to bridge scales and disciplines, to work together to achieve the necessary mindset of ecologically bounded technology.
For us, that has meant learning how to learn from each other: for a designer to learn to see the microscopic scale and grow bacteria in the lab, and for a biologist to learn to see at the scale of social context. Without the tools to collaborate from petri dish to product to system we run the risk of creating a “disruptive” technology that doesn’t disrupt anything.
As biological technologies emerge and scale, we must nurture not only the cells in our petri dishes and fermentation vats, but also the people, organizations, and systems that are necessary to grow a new kind of industry, scaled to our planetary context. A truly radical bio-industrial revolution will see the line between pastoral farmlands, biological foundries, and industrial factories completely blurred. In this new revolution, biology becomes both the creator and constrainer of scale, empowering everyone on the planet to live well—within limits.